There is a glaring gap in our knowledge of the physical world: none of our well-established theories describe gravity’s quantum nature. Yet physicists expect that this quantum nature is essential for explaining extreme situations such as the very early universe and the deep interior of black holes. The need to understand it is called the problem of “quantum gravity.”
The established classical concept of gravity is Einstein’s general theory of relativity. This spectacularly successful theory has correctly predicted phenomena from the bending of light and the orbit of Mercury to black holes and gravitational waves. It teaches us that the geometry of space and time—spacetime—is determined by gravity. So when we talk about the quantum behavior of gravity, we’re really talking about the quantum behavior of spacetime.
We don’t currently have an established theory of quantum gravity, but we do have some tentative theories. Among them, loop quantum gravity (which one of us, Rovelli, helped to develop) and string theory are two leading contenders. The former predicts that the fabric of spacetime is woven from a network of tiny loops, whereas the latter posits that particles are fundamentally vibrating strings.
On supporting science journalism
If you’re enjoying this article, consider supporting our award-winning journalism by subscribing. By purchasing a subscription you are helping to ensure the future of impactful stories about the discoveries and ideas shaping our world today.
Testing these theories is difficult because we can’t study the early universe or black hole interiors in a laboratory. Physicists have mostly assumed that experiments that could directly tell us something about quantum gravity require technology that is many years away.
This situation might be changing. Recent developments suggest it may be possible to perform laboratory experiments that will reveal something about the quantum behavior of gravity. This potential is extremely exciting, and it has raised real enthusiasm among theoretical and experimental physicists, who are actively trying to develop the means to carry out the investigations. The proposed experiments could test the predictions of quantum gravity theories and provide support for the assumptions they’re based on.
The experiments all involve events happening at low energies, where the predictions of strings, loops, and the like agree, so they aren’t going to tell us which specific theory of quantum gravity is correct. Still, experimental evidence that gravity is actually quantized would be groundbreaking.
We already have plenty of observations about gravity’s effects on the quantum behavior of matter. Albert Einstein’s theory works fine in these situations, from stellar dynamics, to the cosmological formation of galaxy clusters, all the way to laboratory experiments on the effect of Earth’s gravity on quantum systems. But in all these scenarios, gravity itself behaves in a way that is consistent with classicalphysics; its quantumfeatures are irrelevant. What’s much more difficult is to observe phenomena in which we expect gravity to behave quantum mechanically.
We both have worked on quantum gravity throughout our careers—Rovelli as a physicist and Huggett as a philosopher. We are keenly interested in exploring what these experiments can and cannot tell us about quantum gravity. If they come to fruition, we might be able to see, for the first time, space and time themselves being quantum.
The two of us were discussing the developments recently during a break at a conference. Over coffee in a café in Oxford, England, we came up with a simple thought experiment illustrating how the quantum nature of gravity could be revealed. (Related ideas have been discussed previously by, for instance, Alejandro Perez of Aix-Marseille University in France, in work on dark-matter detection, and Netanel H. Lindner and Asher Peres of the Technion–Israel Institute of Technology.)
Our idea involves “interference,” which has been crucial in unraveling many aspects of quantum mechanics. Interference is a phenomenon that applies to waves, quantum or not. All waves have a pattern of crests and troughs; the distance between two crests or troughs is the wavelength. If the crests of two waves meet at a point, they combine to produce a crest twice as high as either alone, and when two troughs meet, you get a trough twice as deep. This kind of interference is said to be constructive. Destructive interference, then, is when a wave and a trough overlap and cancel each other out.
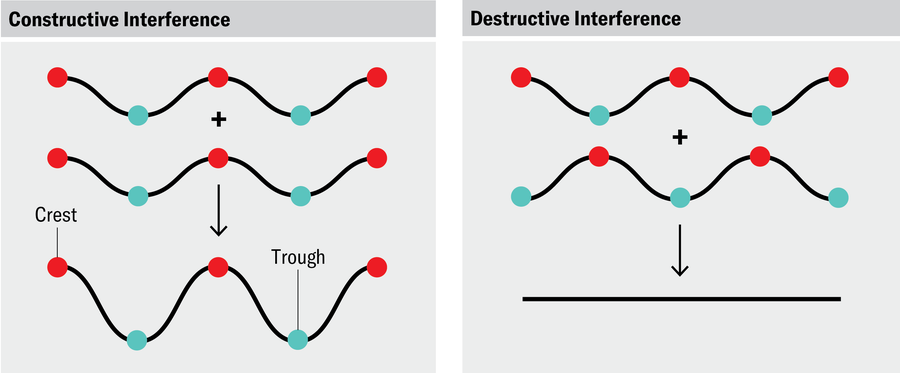
In the 19th century, interference allowed scientist Thomas Young to demonstrate that light acts like a wave. He shined light through two narrow slits to cast an image on a screen behind them. Waves from each slit travel the same distance to reach the point directly between the two slits, so their peaks hit that point at the same time, and they produce constructive interference—that’s where Young saw the brightest light. At points farther along the wall to the right of the light source, the wave from the left slit has to travel a slightly longer distance than the wave from the right, so crests and troughs no longer line up, and the height of the added waves decreases. Eventually there is a point at which the wave from the left has to travel half a wavelength farther than the one from the right, and crests line up with troughs to make destructive interference; here Young saw no light. This pattern, known as “Young’s fringes,” repeated along the wall and showed that light is, in fact, a wave.
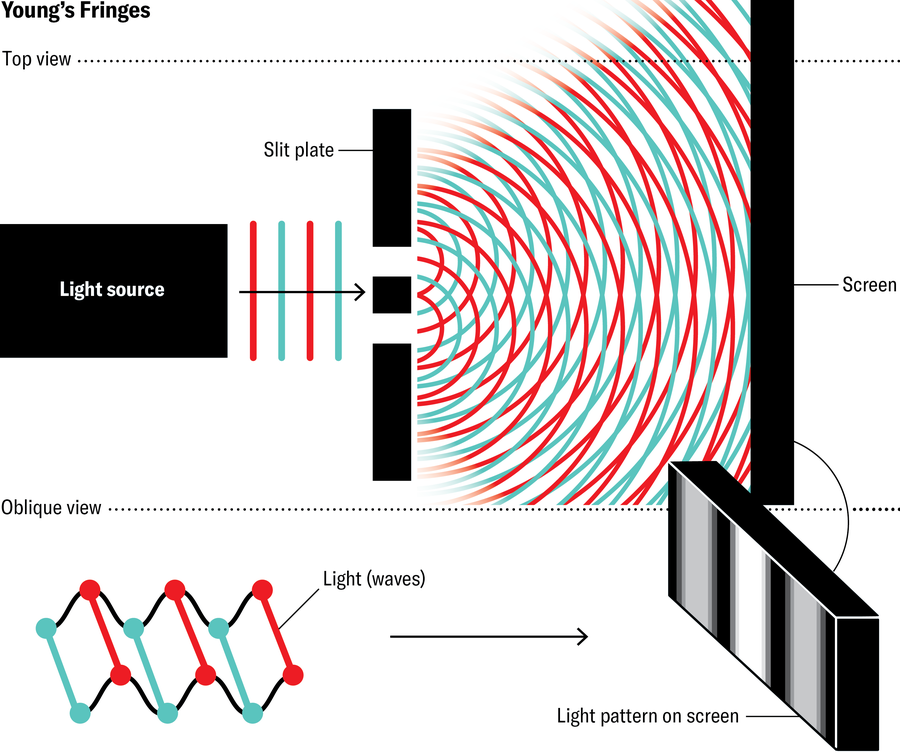
Young’s experiment was purely classical, but variations on this setup became important for quantum physics. In 1923 physicist Louis de Broglie proposed that quantumobjects may behave not like little billiard balls, as they had often been thought of, but like waves. If so, particles such as neutrons should also produce a pattern of fringes in a double-slit experiment—and indeed they do, as demonstrated in the 1980s with neutrons produced in a nuclear reactor.
Amazingly, these experiments produce the same results when neutrons pass one at a time through the double slits. Even a singleneutron sent through the experiment will create interference, meaning it somehow interferes with itself. That can happen only if the neutron acts like two waves that follow two different paths. Because the idea of being in two places at once is so alien to classical particles, a new term was adopted; we say the neutron is in a “superposition” of being both here and there.
Does this part of quantum weirdness apply to gravity? Does it apply to space and time? To address these questions, we turn to general relativity, which tells us the presence of mass (or energy more generally) means that nearby spacetime will be curved. This curvature, in turn, means that objects will be naturally deflected toward mass, explaining its gravitational attraction. Such spacetime curvature also means that clocks run slower when they are closer to a mass. This effect can be used in an interference experiment that brings quantum mechanics and gravity together— a step toward showing gravity is quantum.
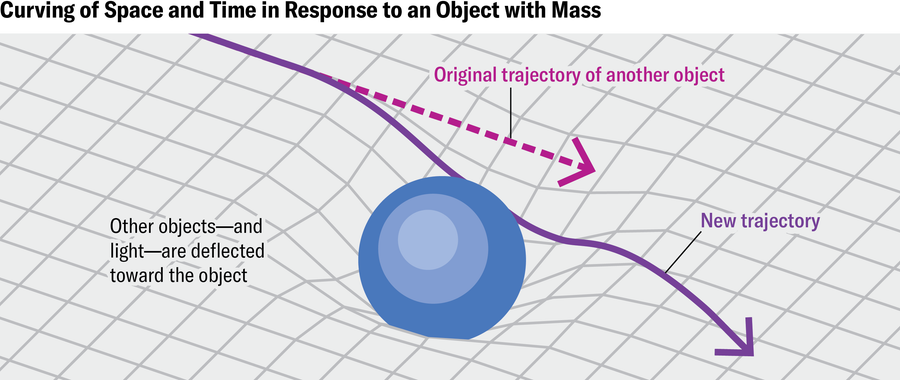
Suppose a neutron, in wave form, is split in two by a mirror that reflects and transmits equal amounts of the wave. The two resulting quantum waves travel different paths to a screen: one travels parallel to the ground and then upward, the other upward and thenparallel to the ground, each path forming two sides of a rectangle. The waves are in sync when they leave the mirror, but because of Earth’s gravity, the wave that follows the lower path will oscillate more slowly, and its crests will arrive slightly after those of the wave that follows the higher path. (The effect of the vertical segment is the same on both.) The result is quantum interference caused purely by the curvature of spacetime.
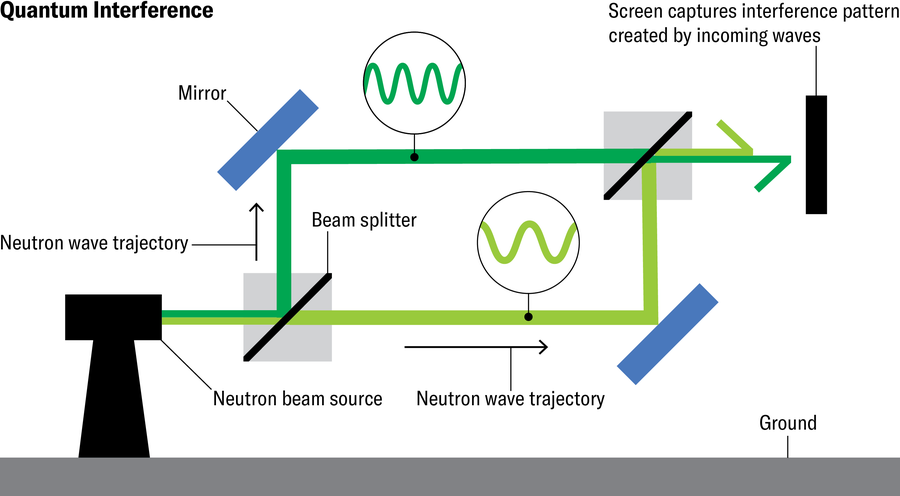
Physicists proposed such an experiment in 1974. The following year Roberto Colella and Albert W. Overhauser, both at Purdue University, collaborated with Samuel A. Werner, then a staff scientist at Ford Motor Company, and successfully carried it out. The team observed the predicted fringe pattern, directly demonstrating the influence of gravity on the quantum behavior of particles, to the great excitement of many scientists. But even though the neutrons in the experiment behaved quantum mechanically, gravity in this case can be described by general relativity, so it is still classical, not quantum.
The breakthrough in the new proposals is that they aim to go further and demonstrate for the first time that gravity, like neutrons and light and all other quantum objects, also has a quantum nature.
According to general relativity, all matter, whether a planet, a speck of dust or a neutron, affects spacetime curvature. The deformation of spacetime produced by a small object is minuscule, but it still happens. But what if a small object is in a quantum superposition of locations? Because each position produces a different spacetime geometry, physicists expect that the result is a quantum superposition of geometries. It is as if spacetime has two shapes at once. It is this quantum weirdness of gravity that we hope to one day see in a laboratory.
The simple thought experiment we came up with that day in Oxford shows how it could be done in principle. Imagine that you shine a light past an object in superposition. That light would travel through a superposition of two spacetime geometries. In one geometry it might be far from the object, in which case the effect of gravity would be negligible, and it would travel in a straight line to a screen. In the other geometry it would pass close enough to the object that gravity would have to be taken into account, so it would follow a curved path to the screen. These two different paths mean that when the waves recombine at the screen, they will interfere and produce the telltale fringe pattern.
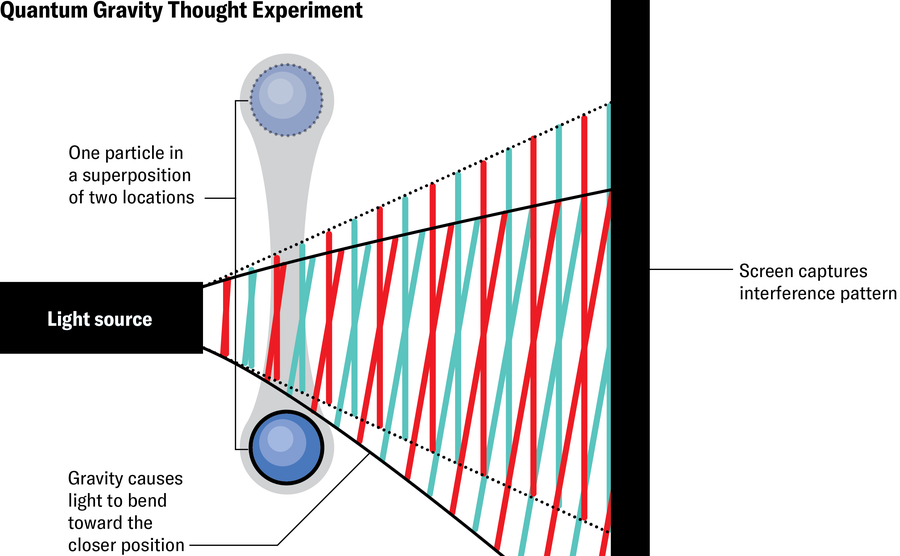
Crucially, interference will not arise unlessgravity can exist in superposition—in other words, unless gravity itself is quantum. If instead gravity is fundamentally classical, no such interference will result. Perhaps, as mathematician and Nobel laureate Roger Penrose has argued, nature picks one of the superposed geometries, causing the mass in superposition to “choose” a single location. Or perhaps there is a single geometry corresponding to a single mass at the average position among its possible locations. Either way, there will be no superposition of geometries, and the light ray will follow a single path and won’t be able to interfere with itself. So if interference fringes were to occur in such an experiment, they would, according to standard physics, show quantumlike behavior of gravity such as a superposition of geometries—a momentous result so far not achieved by any experiment.
What are the prospects of carrying out such an experiment? On one hand, the more massive the object we place in superposition, the greater the effect on gravity and hence on the light. On the other, although every object is fundamentally quantum mechanical, most large, everyday things are essentially impossible to observe in superposition because they interact too much with their environments, hiding any interference. We call this effect “decoherence.” The larger something is, the more chances it has to interact, and the more it decoheres; scientists who have isolated systems to overcome this effect have won Nobel Prizes.
So we are pulled in two directions for our experiment. We need something big enough to let us see gravitational effects but small enough for us to see its quantum nature. We have to find the sweet spot.
Quantum gravity is characterized by three constants of nature: the speed of light, Isaac Newton’s constant describing the strength of gravity, and Planck’s constant describing the scale of quantum phenomena. Arithmetically combining them produces a characteristic “Planck mass” of around 20 micrograms (μg). This is about the same mass as that of a flea egg or a strand of hair a few millimeters long: not large but—unlike the energy involved in the big bang—definitely on a human scale. The sweet spot where we hope to search is plausibly around this mass, which involves both gravitational and quantum mechanical constants.
Recently scientists were able to place an object of that mass into a quantum superposition of locations two billionths of a nanometer apart. This separation, however, is still less than a billionth of the distance we’d need for our tests to have a visible effect. The situation may seem hopeless, but to an experimentalist it sounds like a challenge. Labs are working hard to gain better control over the quantum behavior of Planck-mass bodies and to observe the gravitational effects of masses many times lighter than 20 μg.
If we want to observe a fringe pattern, though, we can’t just shine light at the object in superposition. Even in the gravitational field of a Planck-mass object, the effect will be too small. For us to have any chance of observing what we seek, the light would need a wavelength of 10−32 meter—once again in the inaccessible realm found only at the big bang.
What if, instead of light, we used a second quantum mass to travel near the original mass and exploited its quantum wave nature? The heavier the mass, the greater the gravitational force—and the slower it moves, the longer the mass has to experience that force. These two effects are dramatic: fringes should be observable if the two masses are one ten-thousandth of the Planck mass, tantalizingly close to current experimental ability.
In 2017 a pair of papers about another way of measuring quantum gravity effects in the lab triggered considerable excitement among physicists. The research suggests a strategy for observing a superposition of spacetime geometries that is more subtle and possibly within even closer reach than the one the two of us came up with. Both build on recent advances in theory and experiment that have brought gravity and quantum physics closer together. Both take inspiration from theoretical physicist Richard Feynman’s 1957 version of an idea originally proposed by Soviet physicist Matvei Bronstein.
Start with two Planck-mass particles, each in a quantum superposition of locations. Combined, the pair is in a superposition of four possibilities: one where they are close together, two where they are (much) farther apart and one in which they are at the greatest distance from one another in the experiment. Because the geometry of spacetime depends on the distance between the particles, the different possibilities for the particles’ arrangement correspond to different geometries. Once again, the particle superposition means that gravity, too, is in a quantum superposition.
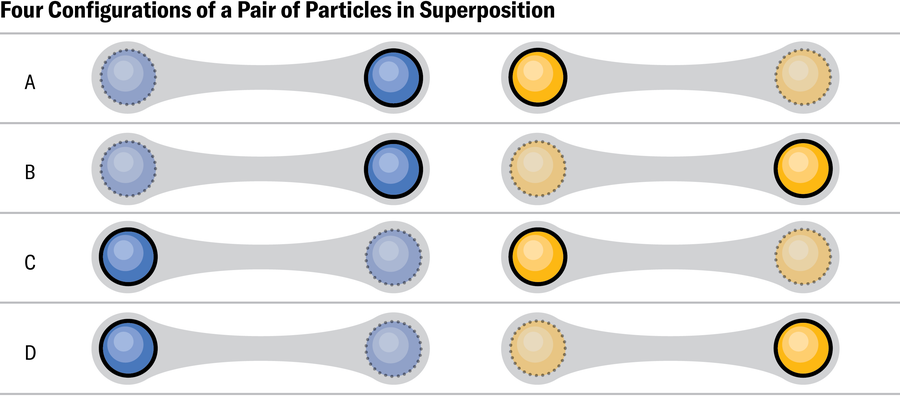
According to quantum theory, a stationary quantum particle is a wave that oscillates with a frequency that depends on its energy, so it is a kind of clock. But as we mentioned, gravity affects the rate at which clocks run. In particular, the particles oscillate at different rates in their different arrangements: the closer they are, the slower they oscillate. As a result, the superposed arrangements get out of phase with one another. As before, when waves get out of phase, they experience interference, which in this case can be measured in characteristically quantum correlations between the two particles called “entanglement.”
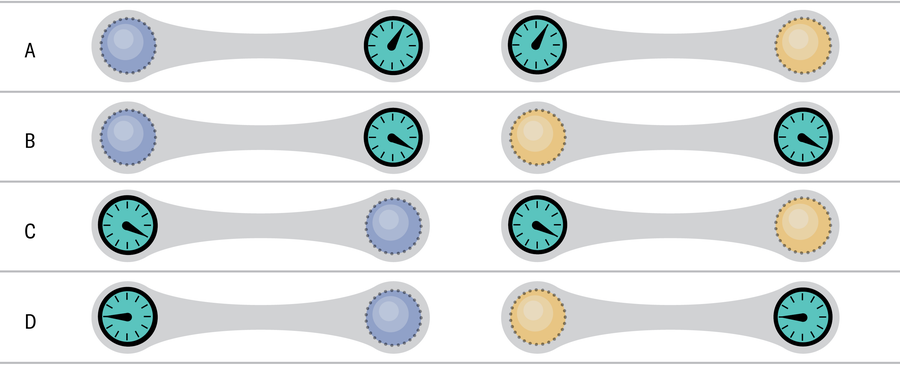
A basic result from the theory of quantum information indicates that entanglement can’t be observed unless the gravitational field through which the particles interact is in a quantum superposition. Therefore, observing the entanglement of the two particles is another means of demonstrating the quantum mechanical behavior of the gravitational field. In 2019 Rovelli published a paper with Marios Christodoulou of the Institute for Quantum Optics and Quantum Information Vienna (IQOQI) arguing that if gravity were indeed caused by deformations of the spacetime geometry, then measuring such entanglement would provide evidence that spacetime geometry can be put into superposition—that space and time, one may say, are quantum.
The 2017 proposal, and this convergence of spacetime physics with the field of quantum information, has caused a splash of experimental, theoretical and philosophical consequences. We are both members of a research consortium called Quantum Information Structure of Spacetime (QISS) that is working to elaborate theoretically and experimentally on these ideas. For instance, a group at IQOQI has been developing the experimental techniques that will be necessary for the entanglement experiment. Other groups in QISS have clarified the theoretical and philosophical significance of the experiment and proposed alternatives to measuring entanglement.
That the QISS collaboration involves philosophers such as Huggett may seem surprising. But there is a tradition of philosophical investigation of space and time that can be traced from antiquity through 17th-century polymaths Newton and Gottfried Wilhelm Leibniz, 19th-century scientist Henri Poincaré, Einstein, and many others. When foundational notions such as space and time need to be rethought, we need people who can bring in a high level of analytical and conceptual—that is, philosophical—clarity. For instance, Huggett recently explored the implications of gravitational entanglement in a book written with science philosophers Niels Linnemann and Mike D. Schneider.
This is not the first time scientists have envisioned laboratory experiments meant to test possible quantum gravity phenomena. But all past proposals, as far as we can tell, involved either unobservably small or extremely speculative effects that aren’t actually predicted by plausible hypotheses about quantum gravity. Rovelli remembers his surprise at first encountering the idea for the new gravity-induced entanglement experiment: a phenomenon that may well become testable and that we expect to be real.
There is still a long way to go over the next few years to carry out such trials (and there would be an even longer path toward enacting our own thought experiment). But if they can be successfully accomplished, they will test the low-energy domain on which almost all theories agree. If researchers find evidence for spacetime in superposition, then they will have the first direct evidence for the basic assumptions of our theories of quantum gravity. We will substantially rule out the possibility that gravity is classical, a significant and previously unexpected step forward. More than that, experimentalists would have reached a new horizon of the physical world, producing a region of spacetime that is observably quantum in a macroscopic laboratory. At last physics will have concretely entered a realm that for now remains a land of hypothesis.
If signs of superposition are notobserved, the experiments will instead support speculations that gravity is intrinsically classical, confounding the expectations of much of the physics community and plunging a huge amount of work from the past 40 years into crisis. Such a result would require a significant revision of our understanding of the world and of the connection between quantum theory and gravity.
In either case, the effect would be momentous.